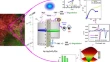
Overview
Journal of Nanostructure in Chemistry (JNC) reports breakthrough research in all aspects of nanoscience at the interfaces of chemistry, biology, biotechnology, materials science, physics, and engineering. Journal of Nanostructure in Chemistry publishes high-quality research, perspectives, and review articles. With our broad scope, we welcome research from all areas across the natural sciences, biomedicine and engineering. Topics covered in the journal include, but are not limited to the following:
- Target drug and gene delivery
- Tissue engineering and regenerative medicine
- Cancer therapy
- Diagnosis and Bioimaging
- Electrochemical detection and sensing
- Food industry and packaging
- Environments (catalyst, coatings, and water treatment)
- Energy (fuel cells, capacitor, laser)
- Director-in-Chief
-
- Omid Moradi
- Impact factor
- 10.1 (2022)
- 5 year impact factor
- 9.4 (2022)
- Downloads
- 250,757 (2023)
Societies and partnerships
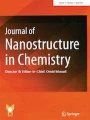
Latest articles
Journal updates
Journal information
- Electronic ISSN
- 2193-8865
- Abstracted and indexed in
-
- Baidu
- CLOCKSS
- CNKI
- CNPIEC
- Chemical Abstracts Service (CAS)
- Dimensions
- EBSCO
- Gale
- Google Scholar
- INIS Atomindex
- Naver
- OCLC WorldCat Discovery Service
- Portico
- ProQuest
- SCImago
- SCOPUS
- Science Citation Index Expanded (SCIE)
- Semantic Scholar
- TD Net Discovery Service
- UGC-CARE List (India)
- Wanfang
- Copyright information